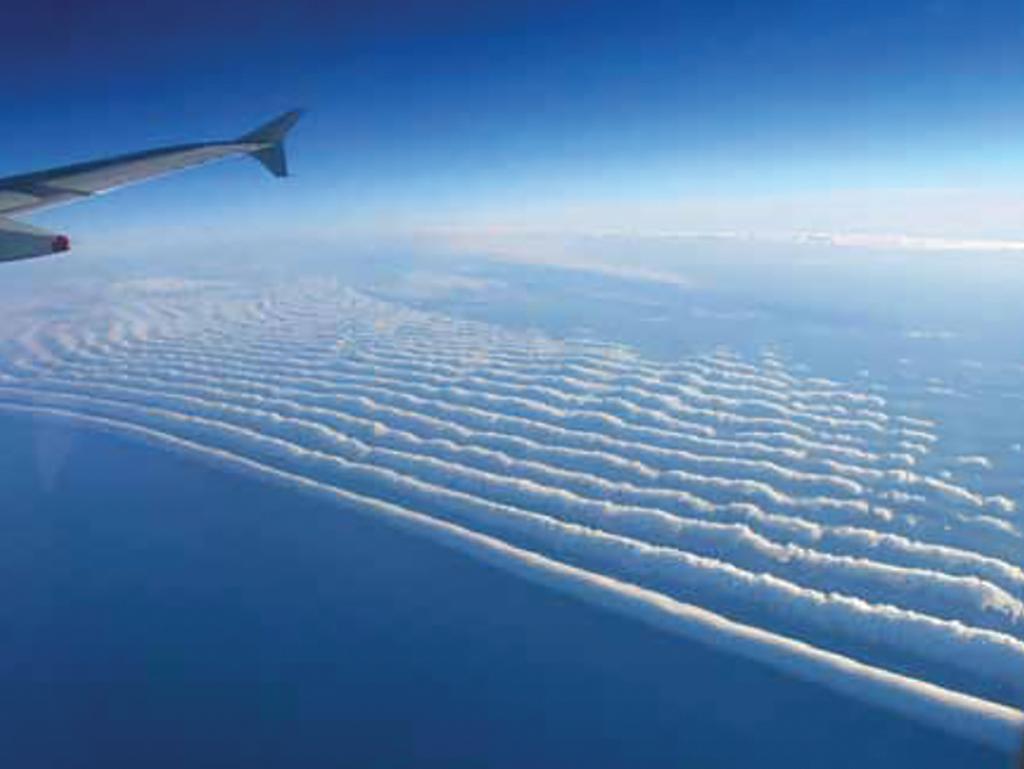
With a wingspan of nearly 200 ft. and max takeoff weight in excess of 700,000 lb., a Boeing 747-100 is not easily disturbed by turbulence. Imagine then being at the controls of a heavily loaded one during departure from Anchorage International Airport (PANC) on March 31, 1993, when extreme turbulence abruptly rolled the aircraft 50 deg. to the left, followed by a significant yaw. Several pitch-and-roll oscillations followed as the pilots struggled to maintain control of the lumbering giant.
The severe turbulence induced dynamic lateral load that so exceeded the load-carrying capability of the No. 2 engine pylon that it ripped completely from the jumbo. The flight crew declared an emergency, dumped a lot of fuel and returned to PANC for an emergency landing.
The NTSB’s investigation determined that the strong rolling motions induced by the atmospheric turbulence produced “multi-axial” loading that caused structural failure of the pylon.
That wasn’t the first time that a rugged commercial airliner experienced severe structural failure due to atmospheric turbulence. On March 5, 1966, British Overseas Airways Corp. Flight 911, a Boeing 707, departed Tokyo’s International Airport destined for Hong Kong. The flight crew requested a visual meteorological conditions (VMC) climb westbound via the Fuji-Rebel-Kushimoto waypoints, which would take the Boeing nearer to Mount Fuji, possibly to give the passengers a better view of the landmark. The request was granted.
After takeoff, the aircraft made a continuous climbing right turn over Tokyo Bay, and rolled out on a southwest heading, passing north of Odawara. It then turned right again toward the mountain, flying over Gotenba, at an indicated airspeed of 320 to 370 kt., and an altitude of approximately 4,900 meters (16,000 ft.), well above the 3,776-meter (12,388 ft.) mountain peak.
Winds at the summit of Mount Fuji were measured at 60-70 kt. from the northwest. While flying into the wind, approaching Mount Fuji from the downwind side, the aircraft encountered severe clear air turbulence (CAT) associated with lee waves. Subsequent investigation determined that the vertical stabilizer failed first, which then broke the left-side horizontal stabilizer as it departed in a left and down motion. Shortly thereafter the right wing failed upward and completely separated from the aircraft. The four engine pylons, ventral fin and forward fuselage also failed from a leftward over-stress, and each eventually departed the aircraft during the inflight breakup.

All 113 passengers and 11 crewmembers were killed in the disaster. The official investigation determined the probable cause as, “The aircraft suddenly encountered abnormally severe turbulence which imposed a gust load considerably in excess of the design limit.”
A U.S. Navy A-4 Skyhawk was sent up shortly after the accident to search for the wreckage and encountered extreme turbulence in the accident area. The cockpit accelerometer display registered peak values of +9 and -4g, causing temporary loss of control, and leading the Navy pilot to believe his fighter would also break up in the turbulence. He regained control and landed safely, but the aircraft was grounded for post-flight inspection. Many other aircraft that passed near Mount Fuji that day also reported moderate to severe turbulence.
Atmospheric rotors pose a great hazard to aviation. The World Meteorological Organization’s “Aviation Aspects of Mountain Waves” states the turbulence contained within mountain wave rotors is worse than that experienced by atmospheric research pilots in thunderstorms!
NTSB records from 1990 to 2017 contain 42 accidents in which mountain wave turbulence was a primary contributing factor. Rotor turbulence was so severe in numerous accidents that it caused the inflight break-up of the aircraft. (See “Turbulence Tragedies” sidebar.) Additionally, the extreme rotor turbulence can make aircraft control impossible. A search of the NTSB database found 16 fatal “Loss of Control” accidents attributed to the turbulence during mountain wave encounters.
The FAA’s Airplane Upset Recovery Training Aid states, “Turbulence, when extreme, can lead to airplane upsets, and/or structural damage. These incidents of turbulence can cause large airspeed, altitude or attitude deviations. The aircraft may be momentarily out of control. Severe or extreme turbulence can be associated with clear air turbulence and mountain waves.” It also states, “Moderate turbulence will be experienced 150-300 mi. downwind on the leeward side when the wind component of 25-50 kt. at ridge level. Severe turbulence can be expected in mountainous areas where wind components exceeding 50 kt. are perpendicular to and near ridge level.”

Extreme turbulence is defined as the “aircraft is violently tossed about and is practically impossible to control. Structural damage may occur. Rapid fluctuations of 25 kt. or greater will be experienced. Vertical gusts equal to 50 fps or greater will be encountered. The most frequent locations of extreme turbulence are found in mountain wave rotors and severe thunderstorms.”
And according to the “Aviation Aspects of Mountain Waves,” “rotor turbulence is much more intense in waves generated by larger mountains. Violent sharp-edged gusts exceeding 12 m/s (approximately 2,362 fpm) have been measured in some Sierra waves, and experienced pilots have reported complete loss of control of their aircraft for short periods of time while flying in the rotor areas.”
Some of the earliest scientific data on rotor turbulence came from the Jet Stream Project, overseen by Joachim P. Kuettner, an avid soaring enthusiast who was a scientist at the U.S. Air Force Cambridge Research Center in 1955. The project used B-29 and B-47 aircraft as well as specially designed Pratt-Read gliders built to tolerate 8-10g to study the atmospheric motions within mountain waves. One flight assignment had project pilot Larry Edgar tasked with descending through a “roll cloud” in a spectacular mountain wave near Bishop, California.
As Edgar’s glider descended through 14,000 ft., it encountered a very severe and turbulent gust, extreme yawing and rolling forces. Positive Gs were so extreme as to cause failure of the left wing at the mid-aileron point, producing a high rate of roll to the left. The high negative Gs built up to the point that they caused damage to Edgar’s eyes. Then the left wing failed completely, causing even more rapid rolling. The load factors created were so adverse that the glider’s nose section broke free from the rest of the structure. It is estimated the glider encountered approximately 16g! Despite all that, Edgar managed to bail out of his disintegrating craft and float safely to the ground, having suffered only bruises.
This and other early research projects helped with understanding “classic” mountain waves in which the rotor is present underneath each wave crest. Most of our modern aviation training texts contain pictures of this configuration. These “classic” waves contain what some researchers categorize as Type 1 rotors. Such rotors are frequently recognizable by rough appearing fracto-cumulus cloud lines that form parallel to a mountain range when sufficient moisture exists in the atmosphere. Rotor clouds are constantly forming on the upwind side and dissipating on the leeward side. The transition from the smooth air in the updrafts and downdrafts to the extreme turbulence of the rotor section is often very rapid.
More recent research aided with advanced instrumentation has helped us understand rotors that rise to much higher altitudes. Research pilots with the National Center for Atmospheric Research (NCAR) in Boulder, Colorado, have studied mountainous airflows for several decades with specially instrumented aircraft to measure the turbulence. In a 1992 experiment, the NCAR Sabreliner experienced severe turbulence at 20,000, 30,000 and 39,000 ft. The unit’s Queen Air along with the Sabreliner encountered severe turbulence at all levels below 15,000 ft. They encountered frequent 2-4g gusts, both horizontal and vertical, in rotors. On one occasion a gust produced a 7g load factor on the aircraft. Surface winds in Boulder exceeded 100 kt. in some locations and the NCAR hangar at nearby Rocky Mountain Metropolitan Airport (KBJC) was severely damaged in an infamous Front Range windstorm. The conditions airborne were even more extreme. Two DC-8s flying over the Front Range experienced temporary losses of control due to the turbulence, and one of them suffered structural damage.
A collaborative group of scientists and organizations has sought to better forecast conditions that create this extreme turbulence. The Sierra Rotors Project was the first phase of a coordinated multi-year effort to study atmospheric rotors and related phenomena in complex terrain. It was a joint National Science Foundation funded project involving the Desert Research Institute, Naval Research Laboratory, Universities of Washington, Utah, Wyoming and Arizona State, the British Met Office, the Army Research Laboratory, the Air Force Research Laboratory, along with the NCAR. The second phase of the project was the recently completed Terrain-Induced Rotor Experiment — nicknamed “T-Rex,” which seems fitting given the ferocity of the subject matter being studied.
The team of 60 scientists hoped to improve the understanding and predictability of CAT caused by complex terrain. The knowledge gained should help forecasters predict when and where rotors are most likely to occur and their intensity, as well as the nature of the mountain waves that crest high above the rotors and cause strong turbulence.
The field activities focused on the Owens Valley to the east of the southern Sierra Nevada, which is the tallest and steepest topographic barrier in the contiguous U.S. The ridgeline of the Sierra Nevada reaches an average elevation of 11,500 ft. MSL, and the tallest peaks exceed 13,100 ft. MSL, including the tallest mountain in the contiguous U.S., 14,505-ft. MSL Mount Whitney. In contrast to the tallest peaks, the valley floor lies at an average elevation of approximately 3,770 ft. MSL. This elevation change occurs in less than 5.5 nm, resulting in 30% eastern facing slopes. Soaring enthusiasts and atmospheric researchers have long known that the mountain waves and attendant rotors can reach particularly striking amplitude and strength there.
The researchers took advantage of the newest advances in remote sensing and numerical modeling. On the ground, they probed the atmosphere with radars, lidars (laser-based radars), automated weather stations, wind profilers and balloons. Those aboard special aircraft including a modified Gulfstream V observed the rotors from above and released dropsondes (instruments that contain temperature, wind and other sensors) into the most turbulent areas. Two other aircraft, the University of Wyoming’s Beech King Air 200T and the UK Environmental Research Council’s BAe146, flew at lower elevations, gathering data by aiming special radars into the rotors.
The $81.5 million Gulfstream, owned by the National Science Foundation and operated by the NCAR, is nicknamed Hiaper, for High-performance Instrumented Airborne Platform for Environmental Research. During the T-Rex project it departed from its base at KBJC to California’s Owens Valley for 10-hr. flights during the project’s observation periods.
What follows are some of the project’s more notable findings: The strongest wave events were found to be associated with (1) an upper-level pressure trough along the Pacific Coast with strong westerly flow across the Sierras and (2) a cold or occluded front approaching California from the northwest, in particular in the pre-frontal stage over the Owens Valley. In addition, the jet stream was typically found to cross Oregon or Northern California during strong wave events. The strongest waves were also correlated with strong winds at the mountain crest level, a pronounced inversion layer and large vertical shear in the lower troposphere.
James Doyle, of the Naval Research Laboratory, and Dale Durran, of the University of Washington, in a paper titled “Rotor and Sub-Rotor Dynamics in the Lee of Three-Dimensional Terrain” (Journal of Atmospheric Sciences, December 2007), found that irregularities along the ridgeline of a mountain chain create “sub-rotors” within the airflow that are intensified well in excess of those in the parent rotor. Because of their intensity, Doyle and Durran opine, such sub-rotors likely pose the greatest hazard to aviation.
The Desert Research Institute’s analysis of the data found considerable variation in the behavior of the waves, for reasons that are not yet completely understood. The teams observed a pronounced diurnal variation of the wave and rotor activity, with the maximum wave and rotor strength occurring in the early evening hours. They also noticed lee wave and rotor response is strongly controlled by changes in the upstream ambient wind and stability profiles.
Two types of rotors were observed during the projects. The first was found to be located under the crest of a lee wave, paralleling the topography and its curvature — the classic Type 1.
The second type was signified by a roll cloud that looked like an almost vertical wall. Researchers currently refer to these as Type 2 rotors. These sometimes have a massive roll cloud with a nearly vertical leading edge and tend to form a straight barrier extending the full length of the mountain range. Type 2 rotors may reach heights of 25,000-30,000 ft.
In the words of Kuettner and Rolf F. Hertenstein, associated with the NCAR and Colorado Research Associates, respectively, in their research report titled “Observations of Mountain-Induced Rotors and Related Hypotheses: A Review,” “It is unlikely that aircraft can be designed strong enough to withstand the excessive loads of a fully developed Type 2 rotor.”
At the International Civil Aviation Organization’s Second High-Level Safety Conference in Montreal in February 2015, information from accident and incident investigations revealed that present day encounters with this atmospheric phenomenon may infringe on current aircraft certification envelopes. The subcommittee overlooking certification issues recommended the follow-up include the need for improved ICAO airworthiness, operations and detection equipment provisions in order to further mitigate changing meteorological risks.
The FAA’s Airplane Upset Recovery Training Aid states, “Avoidance of environmentally induced upsets is the best course of action. Pilots should monitor the environmental conditions and avoid high risk situations.” This is mirrored by Flight Safety Australia, a publication of the Australian Transport Safety Bureau, which cautioned, “It is absolutely essential that aviators are aware of the wind and its potential effects on aircraft.” As pointed out in the fatal accident of an Agusta 47G on Aug. 19, 2001, near Mount Archer, Queensland, Australia, such warnings pertain to rotorcraft as well.
What are the early warning indications that pilots should look for in weather reports so they can anticipate and try to avoid rotor encounters? Any NOTAM containing “ACSL” (altocumulus standing lenticular) should be taken seriously as an indication of mountain wave activity, as well as PIREPs observations. Reports of pressure falling rapidly at stations on the lee side of a mountain are also indicative of mountain wave activity. Reports of strong surface gusts, blowing dust and windsocks at opposite ends of the runway showing greatly varying winds are classic signs of mountain wave turbulence that extends to the surface.
Furthermore, avoid flight in or near rotor clouds as you would a mature thunderstorm. And avoid flight in the vicinity of ragged or frayed lenticular clouds, as these are prime indicators of severe turbulence. Whenever near a rotor, observe the turbulence penetration speeds published in your aircraft’s AFM.
When those of us who enjoy wave soaring see those “lennies” in the air, we dream of the silky smooth updrafts that can lift us to high altitudes, but we also know that extreme turbulence in the rotor and the downdrafts are very severe hazards to all aircraft. Like any significant weather threat, this one demands respect and a knowledge of how to safely operate around it. This is a threat that can’t be shrugged off with a “we fly jets so the weather doesn’t affect us” attitude. There is only one method for dealing with this atmospheric threat, and that is “avoid . . . avoid . . . avoid.”
Turbulence Tragedies: Three Incidents Recounted
Dec. 10, 2015, Hurricane, Utah
Aircraft: RV-7
Injuries: 2 Fatal
The ATP was conducting a local personal flight. Witnesses observed airplane debris floating in the air. Post-accident examination revealed extensive damage to the horizontal stabilizers, elevators and wings consistent with overloading. A review of the weather information indicates that there were likely low-level winds gusting from 26 to 46 kt. at the time of the accident and that moderate to severe turbulence likely existed at the accident site. The NTSB determined the pilot’s abrupt flight control inputs in severe winds and turbulence resulted in an inflight breakup.
May 11, 2005, Ouray, Colorado
Aircraft: Cessna T210
Injuries: 4 Fatal
The airplane was reported missing and the Civil Air Patrol located the wreckage near Mount Whitehouse. National track analysis program radar data depicted the accident flight’s altitude varied from 17,500 ft. MSL to 19,200 ft. MSL. The aircraft ground speed during this time was measured to vary between 124 kt. and 314 kt. An airman’s meteorological information (AIRMET) for occasional moderate turbulence below FL 180 was valid. In addition, an AIRMET for occasional moderate turbulence between FL 180 and FL 410 and possible mountain wave action had been issued. The NTSB determined the probable causes of this accident were the pilot’s inadvertent flight into adverse weather conditions, loss of control and resulting exceedence of the design stress limits of the aircraft, which led to an inflight structural failure. Factors in the accident included the severe turbulence and the mountain wave.
Aug. 19, 2001, near Mount Archer, Queensland, Australia
Aircraft: Agusta 47G
Injuries: 1 Fatal
According to the Australian Transport Safety Bureau, the pilot of an Agusta 47G was fatally injured when he lost control of the helicopter after encountering severe mountain turbulence on the northeast slope of Mount Archer. “The extensive damage to the helicopter, severed tailboom and the location of parts on the ground led transport safety investigators to conclude that the main rotor blade may have contacted the tailboom in flight. This type of damage was consistent with flying into mountain wave turbulence, and may have occurred from one of two events: blade flapping (divergence of the main rotor blade from its normal plane of rotation encountered during severe turbulence) or the pilot’s instinctive reaction to pull up after a sudden nose-down pitch . . . . Weather conditions at the time were conducive to mountain waves.”